Power Measurement Errors
Published by: Mi-Wave© (Millimeter Wave Products Inc.)
Authored by: Lokesh Saggam, Shashi Saggam
Introduction
Power level is frequently the critical factor in the design, evaluation, purchase and ultimately, the performance of almost all RF products and equipment.
When a customer purchases a product with specified power performance for a negotiated price, the final production-line test results must agree with the customer’s incoming inspection data. The receiving, installing, or commissioning phases often occur at different locations, sometimes across national borders. The various measurements must be consistent within acceptable uncertainties.
Measurement uncertainties cause ambiguities in realizable performance of a transmitter. For example, a ten-watt transmitter costs more than a five-watt transmitter. Twice the power output means coverage for twice the geographical area (or 40 percent more radial range for a communication system). Yet, if the overall measurement uncertainty of the final product test is on the order of ±0.5 dB, the manufactured unit could have output power as much as 10% lower than end-user expectations, resulting in lower headroom in its operating profiles.
Signal power level is so important to the overall system performance that it is critical to also consider the power requirements when specifying the components that comprise the system. Each component of a signal chain must receive the proper signal level from the previous component and transmit the proper level to its successor. Power is so important that it is frequently measured twice at each implementation level: once by the vendor, and again at the incoming inspection stations before beginning the next assembly stage.
At the higher operating power levels, each decibel increase in power level becomes more costly in terms of complexity of design, expense of active devices, skill in manufacture, difficulty of testing, and degree of reliability. The increased cost per dB of power level is especially true at microwave frequencies, where the high-power solid-state devices are inherently more expensive and the guardbands designed into the circuits (avoiding maximum device stress) contribute to price increases.
Many systems are continuously monitored for output power during ordinary operation. This large number of power measurements and their importance dictates that the measurement equipment and techniques be accurate, repeatable, traceable, and convenient. The goal of this application note is to guide the reader in making those measurement qualities routine.
Many of the examples cited above used the term “signal level,”and the natural tendency might be to suggest measuring voltage instead of power. At low frequencies, (below about 100 kHz) power is usually calculated from voltage measurements across a known impedance. As the frequency increases, the impedance fluctuates and diminishes the accuracy of power measurements, forcing engineers to create theoretical, calculated voltage and current parameters. Paradoxically, the need for accurate measurements increases simultaneously.
At frequencies from about 30 MHz and up through the optical spectrum, the direct measurement of power is easier and more accurate. Another example of decreased usefulness is in waveguide transmission configurations where voltage and current conditions are more difficult to define.
Methods of Power Measurement
There are three popular methods of sensing and measuring average power at RF and Millimeter Wave frequencies. Each method uses a different kind of device to convert the RF power to a measurable DC or low-frequency signal.
1. Method 1: Using Thermistor
2. Method 2: Using Thermocouple
3. Method 3: Diode Detector
Each method has its own advantages and disadvantages.
Thermocouple:
• True RMS measurement
• Relatively insensitive to temporary overpowering.
• Moderate dynamic range (typically 40dB)
Slow Diode (CW):
• True RMS measurement in the square root area.
• Very sensitive to temporary overpowering.
• Very high dynamic range (typically 90dB)
Faster Diode (Peak):
• True RMS measurement in the square root area.
• Very sensitive to temporary overpowering.
• Moderate to high dynamic range (typically 40 – 80dB). Very Fast
The general measurement technique for average power is to attach a properly calibrated sensor to the appropriate transmission line port. The output from the sensor is connected to a compatible power meter. The RF power to the sensor is turned off and power meter is adjusted to read zero power. Power is then turned on; the sensor reacts to the new input power level, sends a signal to the power meter, and new meter reading observed.
Ideally, the power sensor absorbs all the power incident upon the sensor. In reality, however, the most prevalent errors associated with power sensing are due to the probable impedance mismatch between the characteristic impedance of the RF transmission line and the RF input impedance of the sensor. Also, RF power might be dissipated in places other than in the power sensing element. Such losses are not metered. This defect is measured by the effective efficiency.
Power Sensor Errors
The most prevalent errors associated with the power sensor are the mismatch loss and mismatch uncertainty discussed in the previous paragraphs. A second source of error is the imperfect efficiency of the power sensor.
Effective Efficiency
For most electrical components, efficiency is defined as the ratio of useful output power to total input power. In using a power sensor, the “power in” is the net power delivered to the sensor. It is the incident power minus the reflected power. But not all the power is dissipated in the element of the power sensor.
Some might be radiated into space or leaked into the instrumentation. Some is dissipated in the conducting walls of the power sensor, in a capacitor, or in several other places that are not metered by the instruments.
In order to be metered, the dissipated high frequency power must go through some sort of conversion process to an equivalent DC or low frequency level. There are errors associated with the substitution process. In thermistor sensors, errors result from the fact that spatial distributions of current, power, and resistance within the device itself are different for DC and RF power.
To address both, the usual parasitic losses, as well as the DC/ low frequency substitution problem, a special term, “effective efficiency” has been adopted for power sensors.
• Power Meter Calibration
Multiple standards and processes are involved in calibration of a power meter. Each can be influenced by various factors like personnel carrying out the calibration or environmental factors such as temperature and humidity that contribute to measurement uncertainty.
• Power Meter Uncertainty
Instrumentation uncertainty is the result of a combination of factors such as meter tracking errors, circuit nonlinearities, range-changing attenuator inaccuracy, and amplifier gain errors. It also includes very small sources of uncertainty arising from things like the thermoelectric voltage introduced by temperature gradients within the electronic circuits and interconnecting cables. Even the operator’s subjective interpretation of the meter indication contributes to inaccuracies! This accumulated uncertainty is usually mitigated by the instrument manufacturer, who designs the devices to produce results that contain an acceptable margin of error.
Power Meter Drift
Drift, or long-term stability, is the change in meter indication over a long time (usually one hour) for a constant input power, temperature and line voltage, and is mostly sensor induced. In most cases, drift is actually a drift in the zero setting. For measurements on the upper ranges, drift contributes a very small amount to total uncertainty. On the more sensitive ranges, it can be reduced to a negligible level by zero setting immediately prior to making a reading.
• Sensor Calibration Factor
The calibration factor (Kb) is a combination of the power sensor’s effective efficiency and mismatch loss. Accurate measurement of Kb is complicated and performed mainly by standards laboratories and manufacturers. Most modern power meters can correct their displays by setting a dial or keying in a digital number to the proper Kb value. The uncertainty of Kb (stemming from inaccuracies in measurement of Kb by the manufacturer, NIST, or standards laboratories) is specified by the calibration supplier.
• Power Sensor Linearity
Linearity in power measurement is a characteristic of the sensor. Deviation from perfect linearity usually occurs in the sensor’s higher power range or near the sensor’s specified limits. For thermocouple sensors, linearity is negligible except for the top power range of +10 to +20 dBm, where the deviation is specified at ±3 percent.
• Power Meter Zero Set
In any power measurement, the meter must initially be set to zero with no RF power applied to the sensor. This is accomplished within the power meter by introducing an offset voltage that forces the meter to read zero. The offset voltage is contaminated by several sources including sensor and circuit noise. On higher power ranges, error in zero setting is small in comparison to the signal being measured.
• Power Meter and Sensor Noise
Noise (short-term stability), arises from sources within the power sensor and circuitry. It is specified as the change in meter indication over a short time interval (usually one minute) for a constant input power, temperature and line voltage. One cause is the random motion of free electrons due to the finite temperature of the components. The power observation might be made at a time when this random fluctuation produces a maximum indication, or perhaps a minimum.
Simple Techniques for Reducing Mismatch Uncertainty in Wave Transmission Lines
Several practical techniques reduce mismatch uncertainty. The first involves selecting test equipment with the lowest SWR specification. In this case, controlling mismatch uncertainty is as simple as reducing the reflection coefficient on any transmission lines or components that are part of the test arrangement. Other steps that can be taken to ensure ideal test system performance include:
• Minimize the cable length and number of adapters.
At lower frequencies (e.g., lower than 300 MHz) shorter transmission lines reduce the changes of phase with frequency. For higher frequencies, this method is not viable, because even short lengths of cable form significant fractions of a wavelength. Good quality cable should also be used. If testing multiple devices, the connectors should be designed for hundreds of connect and disconnect cycles. Additionally, converting between different families of connectors may be necessary, but the number of adapters should be minimized. Adapters should convert directly and not be stacked. Also, be wary of mating between dissimilar connectors.
• Use a torque wrench.
For consistent tension, apply appropriate torque values. When tightening screw-type connectors, use a torque wrench to avoid over- or under-tightening the connector. This will ensure there is little variation in tightness when another operator takes over.
• Characterize cables, connectors, adapters.
The best way to check the performance of cables and adapters is by using a vector network analyzer and recording the results for comparison at the next regular test station audit. To optimize the performance of precision connectors, clean and gauge them regularly. When a connector is gauged, it is measured with a special dial gauge to ensure it has not been mechanically damaged. A damaged connector can instantly ruin the mated part.
Advanced Techniques for Improving Mismatch Uncertainty
When the performance of a test arrangement is simply not good enough for the job, several more advanced techniques can be employed to improve mismatch uncertainty and in turn, accuracy. These techniques include:
• Improve the test SWR with an attenuator.
The use of an attenuator (pad) to improve the flatness of a transmission line assumes that the return loss of the attenuator is better than the original source or load. The attenuator is usually placed at the end of the line with the worst return loss. To keep the signal level constant at the load, the generator level must be increased, although doing so limits the applicability of this method to the mid-range of power levels.
• Reduce load reflections with an isolator
Isolators are applied at high power levels, where the economic cost of the power lost in an attenuator would be high, and at very low power levels, where the signal would be masked by thermal noise. Isolator components are narrowband devices and are likely to be more expensive than attenuators.
• Use a power-splitting device.
ere, a leveling loop is employed to create an impedance at the Center Point of the splitter. The resulting “generator output impedance” is equivalent to the highly matched microwave resistor in the second arm of the splitter. The leveling loop uses low-frequency feedback to improve the effective source match to the line. This requires a two-resistor power splitter or a directional coupler. The output of the generator is measured on a power meter and the generator is adjusted so that the indicated power is at the level needed. The technique requires a power meter that is better matched than the signal generator, and an accurately matched two resistor power-splitter or directional coupler. As the measurement frequency increases, so too does the importance of maintaining a low SWR on the transmission line. While mismatch uncertainty can never be eliminated, these practical measures will allow the engineer to keep SWRs to a minimum.
Power Measurement Techniques
One accurate and cost-effective instrument for measuring RF power is the RF power meter. Because it is a scalar instrument, incapable of measuring phase, care should be taken to minimize impedance mismatches whose reflections can add in and out of phase with the signal being measured, resulting in greater magnitude uncertainty. Power meters are broadband and not frequency selective, so any unwanted signals are averaged into the desired measurement. The dynamic range of most power meters is 50 to 70 dB. High-end power meters are capable of specialized measurements such as integrated power across a band and triggering for pulsed RF power. For these reasons, power meters are a good choice if the environment is well controlled.
Another instrument commonly used to measure RF power is a spectrum analyzer. With these more complex RF instruments, engineers can measure the individual spectral components across frequency. The absolute power measurement accuracy is not outstanding, typically + 0.5 to + 2.0 dB. However, the relative power accuracy (or amplitude linearity) is excellent. Therefore, calibrating at a given frequency with a known power level, from a signal generator, for example, results in corrected power measurements that are accurate across a dynamic range greater than 100 dB.
High-performance RF instruments, including vector signal analyzers and vector network analyzers, can measure magnitude and phase, offering the potential for greater error correction and measurement accuracy. In addition to the RF power measurements mentioned previously, specialty measurements can be made on pulsed and other complex RF waveforms. This includes pulse profiling, which measures the power across the envelope of an RF pulse, along with the average and peak power of the RF pulse. Complex IQ waveforms are measured in more of a noise-like fashion with measurements such as peak-to-average ratio, and complementary cumulative distribution function (CCDF).
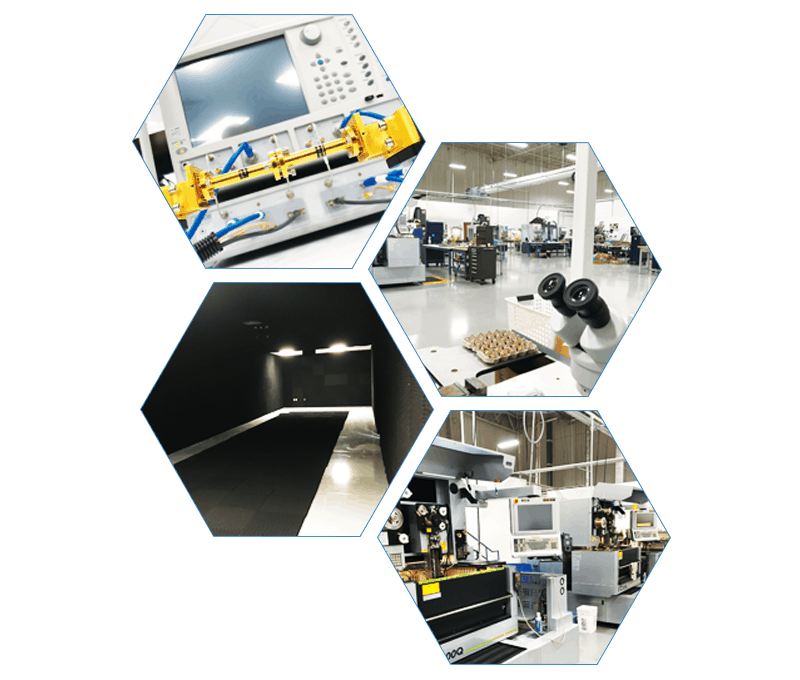
How to Order & Custom Products
As the manufacturing source, we have top sales engineers ready to answer all your questions and quote you on product needs. You’ll find our prices are some of the best since we manufacture all our millimeter wave products in house.
Have a custom job or unique need? No problem! Contact us so we can work on solutions to meet your needs.
2007 Gandy Blvd N
Suite 1310
St. Petersburg, FL 33702
Tel: 727-563-0034
Fax: 727-563-0031
Email: sales@miwv.com